Overview of the Hypothalamus:
The hypothalamus lies centrally at the base of the brain and has a pivotal role in the regulation of homeostasis of many systems in the body for the survival and reproduction in humans (1). The large importance of the hypothalamus is emphasised by the structural organisation and neuronal connectivity it has with all the major brain regions and spinal cord (1). This involves a complex interaction of sensory input from the environment and autonomic, endocrine, and behavioural output to achieve the multitude of functions the hypothalamus is responsible for (2).
Anatomy of the Hypothalamus:
Structure:
The structure of the hypothalamus can be broken down into 3 distinct zones including the medial, lateral and periventricular zone in a medial to lateral orientation (2). The hypothalamus can also be further subdivided into 3 regions including the anterior, posterior and tuberal region in a rostral to caudal orientation (3). Altogether, the hypothalamus is composed of 11 major nuclei groups that each have specific functions across the body by the use of complex feedback mechanisms, secretion of hormones or neurological connections (2, 4). In general, the nuclei located in the medial and lateral zones are for regulating autonomic and somatic behaviour whilst the nuclei in the periventricular zone regulate the endocrine system either through neuronal input to the posterior pituitary, or portal blood vessels to the anterior pituitary (2, 3). The 11 nuclei include the paraventricular, preoptic, anterior, suprachiasmatic, supraoptic, dorsomedial, ventromedial, arcuate, posterior, mammillary, and lateral complex nucleus (Figure 1).
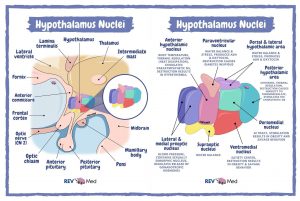
Figure 1. Diagrammatic visualisation of the 11 major hypothalamic nuclei of the hypothalamus relative to surrounding structures (left) and their general location and function (right).
Importantly, the hypothalamus is one brain region that displays a sexually dimorphic structure between males and females. The main nuclei with known sex differences are the arcuate nucleus, the ventromedial nucleus and the medial preoptic nucleus (1, 5). This results in sex differences in sexual behaviours, aggression, parenting as a result of an exposure to different hormone types and levels (5).
Function:
As previously noted, the broad function of the hypothalamus is involved as the integration centre for various bodily functions and processes to maintain homeostatic levels. These are either maintained by neurological connections with the autonomic and somatic systems, but also by hormonal regulation and feedback systems through the pituitary gland (2, 3). The 11 specific nuclei located in their specific zones and regions along with their general function are outlined in Table 1.
Nucleus | Zone | Region | General Function |
Paraventricular | Periventricular, Medial | Anterior, Tuberal | Fluid balance, milk let-down, parturition, autonomic & anterior pituitary control |
Preoptic | Medial, Lateral | Anterior | Lateral anterior thermoregulation, sexual behaviour |
Anterior | Medial | Anterior | Lateral anterior thermoregulation, sexual behaviour |
Suprachiasmatic | Medial | Anterior | Biological rhythms |
Supraoptic | Medial, Lateral | Anterior | Fluid balance, milk let-down, parturition |
Dorsomedial | Medial | Tuberal | Emotion (rage) |
Ventromedial | Medial | Tuberal | Appetite, body weight, insulin regulation |
Arcuate | Periventricular, Medial | Tuberal | Control of anterior pituitary, feeding |
Posterior | Medial | Posterior | Thermoregulation |
Mammillary | Medial | Posterior | Emotion and short-term memory |
Lateral Complex | Lateral | Tuberal | Appetite and body weight control |
Table 1. The 11 hypothalamic nuclei and the region and zone they occupy within the hypothalamus, as well as their general function in terms of homeostasis or regulation. |
Commonalities of development:
The general development of the hypothalamus progresses through similar stages in both males and females in the early stages prior to any hormonal influence. Starting as the neural tube, this differentiates into the three primary vesicles of the embryo: the forebrain, midbrain and hindbrain (2). The forebrain differentiates into the telencephalon and diencephalon, in which the hypothalamus is derived from the diencephalon (Figure 2.) (2).
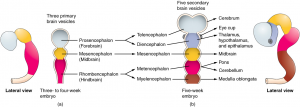
Figure 2. The general development and differentiation origin of the hypothalamus, starting from the growing neural tube (left) through to the primary vesicles and its derivation from the diencephalon (right).
Hypothalamic neurogenesis occurs between embryonic day 10 and 16 in the mouse (6). This progresses through stages such as regionalisation, neuronal stem cell proliferation, differentiation and finally migration (5). However, this process is regulated by many transcription factors working in a precise spatiotemporal pattern (5, 7). For example, FKH5 is essential in the proper development of the mammillary nucleus of the hypothalamus by loss-of-function studies (8). Conversely, SIM1 is essential for the proper structural formation of the paraventricular nucleus and supraoptic nucleus to produce the hormones vasopressin and oxytocin (9).
Known sex differences in hypothalamic development:
The largest known contributing factor in the sexual differentiation of the hypothalamus is the influence of hormones. This is studied in mice, where a hormonal influence is initiated during the perinatal period (around the time just before and after birth) which occurs around E18 (embryonic day 18) in mice (10). This is called the testicular androgen surge in males and is the sensitive period for the organisational effects of androgens on the brain (10).
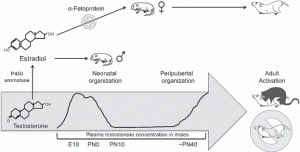
Figure 3. The sensitive period for the organisational effects of steroids/androgens on the brain/hypothalamus between males compared to the females overtime. Peak testosterone seen between E18 (embryonic day 18) to PN0 (postnatal day 0 [birth]).
Therefore, the androgen surge and the presence of alpha-fetoprotein (determined by sex) during this critical period (first 10 days following birth in mice) determines a sex specific hypothalamic development and organisation.
Following aromatisation, estradiol in males acts on estrogen receptors. Transgenic mouse studies using alpha and beta estrogen receptor knockouts (ERα and ERβ) show that ERα is closely associated with masculinisation and is expressed higher in males (10, 1, 5).
As a result, this hormonal influence of estradiol at the time of birth results in several hypothalamic nuclei displaying a high sexual dimorphism. The most well-known are the arcuate nucleus, preoptic nucleus, and the medial basal hypothalamus (1, 5).
Sex differences in the male and female hypothalamus:
The effect of sex chromosome complement on hypothalamic sex differences:
The known link between the developmental exposure of estradiol following the perinatal androgen surge and the sex differences seen in the hypothalamus are noted above. However, studies have looked at the regulation of sex differences in the hypothalamus before this hormonal influence has occurred, by looking at the effect of sex chromosome complement (XX or XY).
This required isolating the effect of sex chromosome complement from gonadal sex, allowing to determine the direct genetic effect of the sex chromosomes and not the effect of the hormones produced by the gonads. The Four Core Genotypes (FCG) mouse model was used, whereby the male gonad-determining gene SRY is relocated to an autosome (13, 14). Therefore, sex differences in the hypothalamus can be determined by the sex-biased effect of the X and Y genes.
It is shown that hypothalamic neurons show sex differences in neuritogenesis (the formation of neurite processes) by a differential expression of neuritogenic genes in males and females (14, 11). This study by Cisternas used E14-16 hypothalamic neurons from the FCG mouse model, meaning that these were obtained prior to the perinatal androgen surge (which occurs around E18). Therefore, with the absence of estradiol, the effect of sex chromosome complement (XX or XY) can be investigated. It showed that female hypothalamic neurons are longer than males simply due to a sex-biased effect of the X and Y genes (14, 11). This was because female hypothalamic neurons have a higher expression of Ngn3 (thus must be regulated by the sex chromosomes), which is a gene implicated in neuritogenesis causing an increased axon length growth (Figure 4.) (11).
However, it was shown that the subsequent addition of estradiol (mimicking the perinatal androgen surge) caused only male hypothalamic neurons to increase their axon length and not females (Figure 5.) (11). It showed that estradiol specifically increases Ngn3 mRNA expression, and therefore neuritogenesis in male hypothalamic neurons (11).
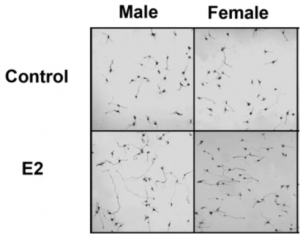
Figure 5. Images of hypothalamic neurons of males vs female, when either treated with a control or 17-β-estradiol (E2) at E14.
Further investigation shows male hypothalamic neurons express higher levels of ERα (alpha estrogen receptors), and that estradiol only acts through ERα to increase Ngn3 expression, and thus form longer axons (Figure 6.) (11, 10, 1, 5). Overall, this shows that male hypothalamic neurons are more sensitive to estradiol, which aligns with the previous knowledge that estradiol drives masculinisation in males. This shows that it is sex chromosomes that regulates the sex differences in the development of hypothalamic neurons prior to gonadal hormonal influence, and corroborates with the known differences in sex biased genes over developmental stages in males and females.
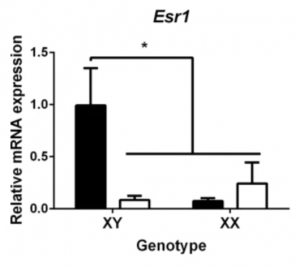
Figure 6. Graph determining the effect of sex chromosome complement (XY or XX) on the level of mRNA expression of ERα and ERβ in hypothalamic neurons.
The effect of epigenetics on hypothalamic sex differences:
Epigenetic sex differences in the hypothalamus are also known. A study by Cabrera Zapata investigated the sex differences of Kdm6a; a demethylase which promotes chromatin accessibility and therefore gene expression (15). It is found to be X-chromosome linked and has been shown to commonly escape X-inactivation in different cell types and developmental stages (11, 15). This results in Kdm6a always having a higher expression in females due to X chromosome dosage (two in females, one in male).
Interestingly once again in mice, Kdm6a causes an increased expression of Ngn3 (Figure 7.). When looking at the previous study, Ngn3 is higher in female hypothalamic neurons prior to any hormonal influence causing females to have longer axons than males (11). Therefore, Kdm6a may explain the reason that females have longer axons in the hypothalamus before brain masculinisation at around E14 (prior to the perinatal androgen surge).
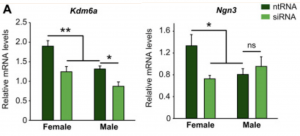
Figure 7. RT-qPCR analysis of Kdm6a and Ngn3 in control (ntRNA) or Kdm6a knockdown (siRNA). This shows that both are expressed higher in females in control conditions, and a Kdm6a knockdown only decreases Ngn3 in female hypothalamic neurons.
Adding further to this, females with high Kdm6a and thus high Ngn3 have an increased expression of POMC neurons and a decreased expression of NPY neurons in the arcuate nucleus of the hypothalamus. However, this is the opposite in males where lower Kdm6a and thus lower Ngn3 favour an increased expression of NPY neurons and a decreased expression of POMC neurons (15). In terms of the function of these two hypothalamic neuron populations within the arcuate nucleus, POMC neurons suppress feeding behaviours, whilst NPY neurons stimulate feeding behaviours (15, 16).
Therefore, since NPY neurons stimulate feeding behaviours and are in a higher expression in males, this may be contributing to a sex bias seen in the prevalence of diabetes and obesity (Cabrera, Pelling). This is further evident with a study showing that deletion of a known transcription factor TAp63 in POMC neurons induces a ‘male-like’ diet-induced obesity in female mice and a decreased POMC neural activity (17).
Therefore in terms of human applicability, sex differences in the epigenetics and gene expression in the development of the hypothalamus may be causing a sex-biased predisposition to obesity and diabetes diseases. By having a greater understanding of the sexually dimorphic processes occurring in terms of a developing hypothalamus leading to a dysfunction in energy homeostasis, this could lead to greater treatments and interventions for obesity which is highly prevalent in males.
Reference List:
- Neigh, G. N., & Merrill, L. (2016). Sex Differences in Neuroanatomy and Neurophysiology: Implications for Brain Function, Behavior, and Neurological Disease. In Sex Differences in Physiology(pp. 17-44). Academic Press
- Bear, M. H., Reddy, V., & Bollu, P. C. (2018). Neuroanatomy, hypothalamus.
- Crivii, C. B., Clichici, S. V., & Filip, A. G. (2021). Anatomy and Topography of the Hypothalamus. The Human Hypothalamus: Anatomy, Dysfunction and Disease Management, 7-14. https://doi-org.ezproxy.otago.ac.nz/10.1007/978-3-030-62187-2_2
- Müller, H. L., Tauber, M., Lawson, E. A., Özyurt, J., Bison, B., Martinez-Barbera, J. P., … & van Santen, H. M. (2022). Hypothalamic syndrome. Nature Reviews Disease Primers, 8(1), 24. https://doi.org/10.1038/s41572-022-00351-
- Fujiyama, T., Miyashita, S., Tsuneoka, Y., Kanemaru, K., Kakizaki, M., Kanno, S., Ishikawa, Y., Yamashita, M., Owa, T., Nagaoka, M., Kawaguchi, Y., Yanagawa, Y., Magnuson, M. A., Muratani, M., Shibuya, A., Nabeshima, Y., Yanagisawa, M., Funato, H., & Hoshino, M. (2018). Forebrain Ptf1a Is Required for Sexual Differentiation of the Brain [Article]. Cell Reports (Cambridge), 24(1), 79–94. https://doi.org/10.1016/j.celrep.2018.06.010
- Shimogori, T., Blackshaw, S., Lee, D. A., Miranda-Angulo, A., Yang, Y., Wang, H., Jiang, L., Yoshida, A. C., Kataoka, A., Mashiko, H., Avetisyan, M., Qi, L., & Qian, J. (2010). A genomic atlas of mouse hypothalamic development [Article]. Nature Neuroscience, 13(6), 767–775. https://doi.org/10.1038/nn.2545
- Xie, Y., & Dorsky, R. I. (2017). Development of the hypothalamus: Conservation, modification and innovation [Article]. Development (Cambridge), 144(9), 1588–1599. https://doi.org/10.1242/dev.139055
- Wehr, R., Mansouri, A., de Maeyer, T., & Gruss, P. (1997). Fkh5-deficient mice show dysgenesis in the caudal midbrain and hypothalamic mammillary body [Article]. Development (Cambridge), 124(22), 4447–4456. https://doi.org/10.1242/dev.124.22.4447
- Xu, C., & Fan, C.-M. (2007). Allocation of Paraventricular and Supraoptic Neurons Requires Sim1 Function: A Role for a Sim1 Downstream Gene PlexinC1 [Article]. Molecular Endocrinology (Baltimore, Md.), 21(5), 1234–1245. https://doi.org/10.1210/me.2007-0034
- Lenz, K. M., & McCarthy, M. M. (2010). Organized for sex – steroid hormones and the developing hypothalamus [Article]. The European Journal of Neuroscience, 32(12), 2096–2104. https://doi.org/10.1111/j.1460-9568.2010.07511.x
- Cisternas, C. D., Cabrera Zapata, L. E., Mir, F. R., Scerbo, M. J., Arevalo, M. A., Garcia-Segura, L. M., & Cambiasso, M. J. (2020). Estradiol-dependent axogenesis and Ngn3 expression are determined by XY sex chromosome complement in hypothalamic neurons [Article]. Scientific Reports, 10(1), 8223–8223. https://doi.org/10.1038/s41598-020-65183-x
- Schwarz, J. M., Liang, S.-L., Thompson, S. M., & McCarthy, M. M. (2008). Estradiol Induces Hypothalamic Dendritic Spines by Enhancing Glutamate Release: A Mechanism for Organizational Sex Differences [Article]. Neuron (Cambridge, Mass.), 58(4), 584–598. https://doi.org/10.1016/j.neuron.2008.03.008
- Arnold, A. P., & Chen, X. (2009). What does the “four core genotypes” mouse model tell us about sex differences in the brain and other tissues? [Article]. Frontiers in Neuroendocrinology, 30(1), 1–9. https://doi.org/10.1016/j.yfrne.2008.11.001
- Scerbo, M. J., Freire-Regatillo, A., Cisternas, C. D., Brunotto, M., Arevalo, M. A., Garcia-Segura, L. M., & Cambiasso, M. J. (2014). Neurogenin 3 mediates sex chromosome effects on the generation of sex differences in hypothalamic neuronal development [Article]. Frontiers in Cellular Neuroscience, 8(JULY), 188–188. https://doi.org/10.3389/fncel.2014.00188
- Cabrera Zapata, L. E., Cambiasso, M. J., & Arevalo, M. A. (2022). Epigenetic modifier Kdm6a/Utx controls the specification of hypothalamic neuronal subtypes in a sex-dependent manner [Article]. Frontiers in Cell and Developmental Biology, 10, 937875–937875. https://doi.org/10.3389/fcell.2022.937875
- Pelling, M., Anthwal, N., McNay, D., Gradwohl, G., Leiter, A. B., Guillemot, F., & Ang, S.-L. (2011). Differential requirements for neurogenin 3 in the development of POMC and NPY neurons in the hypothalamus [Article]. Developmental Biology, 349(2), 406–416. https://doi.org/10.1016/j.ydbio.2010.11.007
- Wang, C., He, Y., Xu, P., Yang, Y., Saito, K., Xia, Y., Yan, X., Hinton, A., Yan, C., Ding, H., Yu, L., Shu, G., Gupta, R., Wu, Q., Tong, Q., Lagor, W. R., Flores, E. R., & Xu, Y. (2018). TAp63 contributes to sexual dimorphism in POMC neuron functions and energy homeostasis [Article]. Nature Communications, 9(1), 1544–11. https://doi.org/10.1038/s41467-018-03796-7