What is the Preimplantation period?
The preimplantation period encompasses all molecular events from egg fertilisation until invasion of the uterine epithelium by the last-stage blastocyst [1]. At the one-cell stage, migration, and fusion of the maternal and paternal pronuclei drives a series of cell replications through cleavage, as shown in Figure 1 [2]. Simultaneously, activation of the embryonic genome occurs through triggering genetic and epigenetic reprogramming. The ongoing cell replications result in a compacted morula to which, the totipotent cells undergo two rounds of asymmetric cell divisions, resulting in expansion from 8 to 32 cells [3]. During the morula stage, the first distinct cell populations arise through differences in polarity, which marks the blastocyst stage. Polar cells situate to the circumference of the morula, closest to the environment, and form the trophectoderm (TE). Later in embryogenesis, the TE induces the development of the placenta after implantation [4]. This coincides with the proliferation of trophoblasts as the first embryonic cell type with highly differentiated functions [5]. On the contrary, apolar cells situate within the middle of the developing embryo and form the inner cell mass (ICM). Within the late pre-implantation blastocyst, cells of the ICM commit to forming the epiblast or primitive endoderm. The epiblast gives rise to the fetus and extraembryonic mesoderm whereas, the primitive endoderm develops extraembryonic endoderm layers of the visceral and parietal yolk sacs [6]. These first cell fate decisions are critical to defining future lineage pathways that make up all parts of the embryo throughout development. The length of the preimplantation period varies between mammalian species. For humans, the time from fertilization to uterus implantation is 7 days whereas, for mice, it’s approximately 5.5 days. Although there is a difference in time related to the developmental stage between mouse and human embryos, the time points relative to cellular size can be adjusted for studying sex-biased expression [7]
Overview of Sex dimorphic expression within the preimplantation period
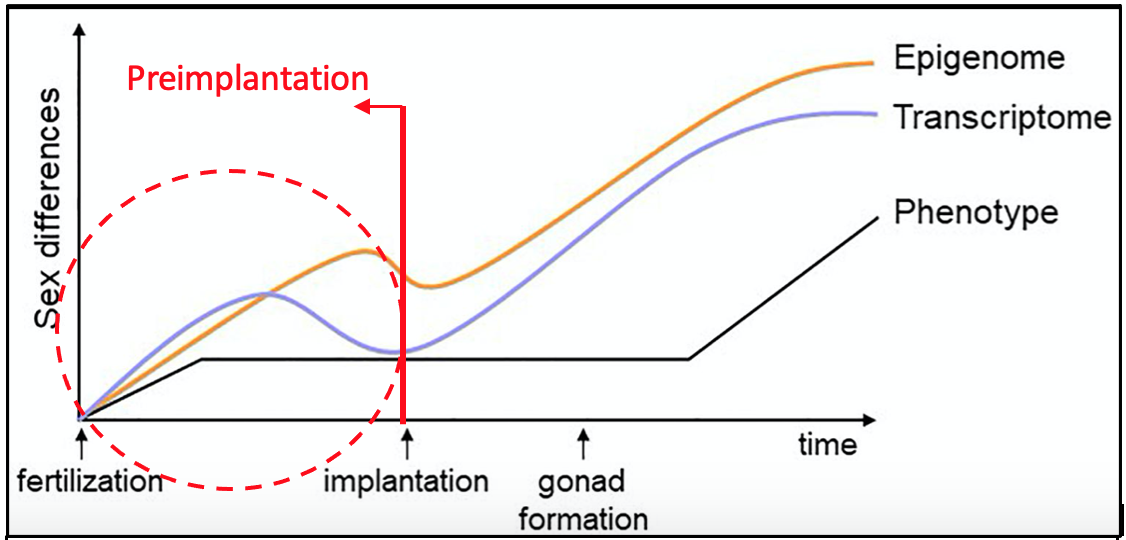
Figure 2. Schematic graph indicating the changes in sex differences over embryonic development events.
Developmental programming refers to changes in the environment of a developing organism that can cause permanent or long-term effects on a phenotypic characteristic carried into adulthood [8]. The composition of sex chromosomes present within this period can drive differential expression of genes defining, distinct regulatory networks between males and females [9]. Expression of transcription factors and epigenetic remodeling factors can form landscapes of expression patterns and construct future pathways for later development, as shown in Figure 2. Traditionally mammalian sex-specific differences were believed to establish from gonad formation and subsequent, regulation by sex hormones [7]. Interestingly, recent research has demonstrated the composition of sex chromosomes to drive sex-biased expression of genes within the pre-implantation period of embryogenesis. While this builds on the traditional view of initial sex-biased gene expression, it departs due to being hormone-independent. Investigating sex-biased expression within the preimplantation embryo has uncovered distinct regulatory networks, driven by sex chromosome complements [10]. The sexually dimorphic expression of genes within these early stages contributes to developmental programming. The composition of the sex chromosomes additionally influences interactions directly and indirectly with autosomal genes that constitute the rest of the genome [9]. Expanding knowledge of molecular systems which regulate these clear differences from individual to individual inevitably, will increase our understanding of developmental disorders, particularly those linked to an individual’s sex
Mechanisms driving sex-specific expression within the preimplantation period
The sex chromosome partner composition determines the expression and regulation of genes present, specific to X or Y [9]. Males have one X and one Y chromosome (XY) whereas, females have two X chromosomes (XX) [10]. The differential expression of genes between XY and XX relates to presence and dosage through regulation. Since females have two copies of the X chromosome, one undergoes inactivation in each cell randomly, to ensure dosage compensation between sexes in mammals [12]. Through inhibitory pathways, X chromosome inactivation (XCI) ensures one copy is silenced per cell through epigenetic modifications and forms a heterochromatic Barr body, as depicted in Figure 3 [13]. Sometimes, genes can escape the XCI process without dosage compensation and lead to higher levels of expression. Usually, escapists aren’t harmful yet, this can alter regulatory mechanisms which affect downstream pathways [14]. The female-specific process of XCI has been suggested to contribute towards a sex-specific developmental delay, relative to males. This suggested pause in female embryonic development has been hypothesised to be a mechanism by which, sex-specific differences enhance. It seems the pause allows females time to generate female-specific regulatory networks and become indifferent alike, for males with an absence of pause in development for XCI.
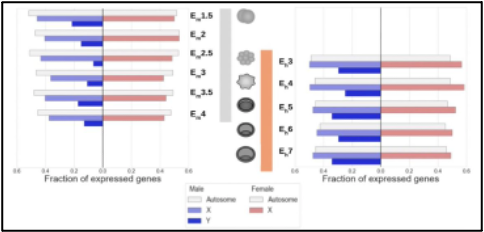
Figure 4. Differential expression of sex-biased genes relative to early embryonic development stage in mice (left) and humans (right).
Ultimately, the presence of XX or XY can alter the presence and expression of different genes. Figure 4 highlights evidence for autosomal and sex-biased expression relative to, the stage of embryo development within mice and humans. Within both mammals, Y-linked expression is lower than expression of X-linked and autosomal genes. However, this is due to the smaller size of the Y-chromosome. Differential gene expression from the X or Y chromosome is attributed to, the interaction of sex-chromosome-specific genes and autosomal genes which have, a relatively constant expression throughout preimplantation. Sex-biased expression is detectable within the autosomes, sex chromosomes, and embryonic stem cells in rodents, bovines, and primates [7].
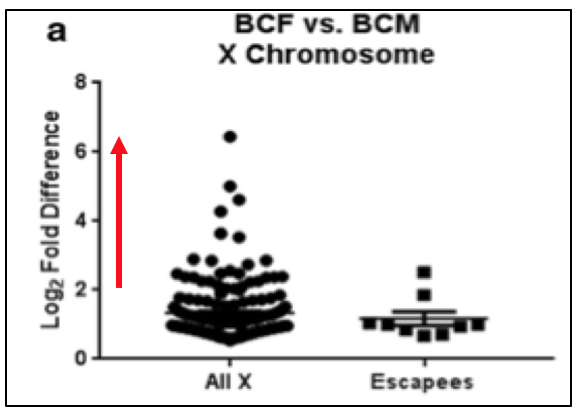
Figure 5. Graph depicts Log2 Fold Difference of X-linked gene expression. Red line indicates ≥2-fold expression.
X-linked genes refer to the exclusive presence of genes on the X chromosome. Since females undergo XCI, these genes are expressed differently in males and females due to dosage. Werner et al. demonstrated, genes escaping XCI in females may be expressed ≥2-fold more than normal levels, as seen in Figure 5. Directly doubling the normal expression would suggest an extra copy. However, greater than 2-fold indicates an additional layer of regulation occurring. Within this study, it was proposed the additional regulation is driven by more active transcription factors. Reasoning for this may be due to, the inheritance of open chromatin from the parental chromosome. Additionally, regulatory polymorphisms could be causing one of the two alleles to have a greater expression by changing the binding affinity and consensus sequences for transcription factors.
Effect of preimplantation sex-specific gene expression on transcriptional regulation
Transcription factors and epigenetic remodeling factors are focused on for studying sex-biased expression in early embryo genesis due to altering development programming. Epigenetic modifications determine the accessibility of the chromatin and further, the ability of transcription factors to activate the expression of genes. Therefore, the epigenetic landscape can determine transcriptional activity. Although, transcriptional sex-biased effects can have a short duration, sex-biased expression of epigenetic enzymes can establish patterns in later stages of development and guide development programming.
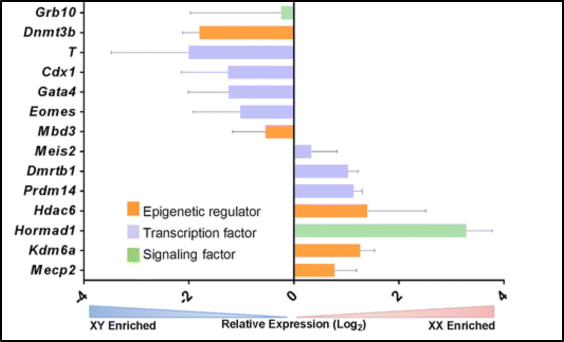
Figure 6. Sex-biased expression (XX or XY enriched) of epigenetic regulators, transcription factors and signaling factors
Within Figure 6, sex-biased expression is evident within epigenetic regulators, transcription factors, and signaling factors [9]. Here, mice embryonic stem cells enriched for XX show higher expression of the signaling factor Hormad1 which is, involved in generating and processing double-stranded DNA breaks during the pairing of homologous chromosomes [15]. Further, gene ontology analysis has discovered notable differences between male and female cells in the early stages of development. The processes of cell organization and compartmentalization were delayed yet, in male cells, RNA and DNA-related processes were delayed [7]. Through conducting single-cell RNA-sequencing Zhou et al., identified gene expression differences at the chromosome level between, 8-cell stage male, and female embryos [16]. Specifically, they showed differences in the transcriptional output and regualtion of genes. The expression of autosomal genes became detectable in later stages of development.
Effect of preimplantation sex-specific gene expression on embryonic stem cell differentiation
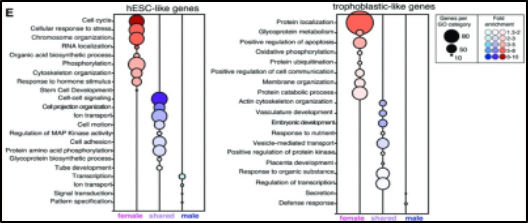
Figure 7. Genes down-regulated in human embryonic stem cells (left) and up-regulated in trophoblastic cells (right) during the differentiation of trophoblastic progenitor cells
Embryonic stem cell differentiation possesses sexually dimorphic expression of genes and is relative to lineage pathways. Syrett et al. demonstrated trophoblastic progenitor cells to differentially expressed genes in vitro. As mentioned previously, trophoblastic cells have critical roles in the formation, growth, and invasion of the placenta after preimplantation [17]. Interestingly, this study utilized human embryonic stem cells (hESCs), with a greater pluripotent state, and further, differentiated them into trophoblastic cells. This portrayed female-specific differentiation to have a greater amount of differentially expressed genes compared to males. Specifically, female cells displayed increased upregulation of placental genes with roles in trophoblast function and differentiation such as ENDOU, SP100, MUC15, CGA, and CGB. However, male differential expression showed up regulation of endogenous placental retroviral genes of ERVV-1, ERVV-2, and HSD3B1, a trophoblast-specific gene. Figure 7 clearly depicts how as trophoblastic differentiation proceeds, female-specific expression of genes increases. Within hESCs female cells down-regulate genes associated with cell cycle, cellular response to stress, and chromosome organization whereas, in trophoblasts genes associated with protein localization, positive regulation of apoptosis, and protein catabolic processes are upregulated. Overall, females and males clearly show statistically significant differences in differentiating trophoblastic cells. The female contribution of which, is critical towards the formation of the placenta. This example depicts how sex-bias expression has a large contribution towards ESC differentiation within different tissues.
The significance of researching preimplantation sexual differences
The coalescence of research focused on the preimplantation period enables a deeper understanding of early embryo development and the mechanisms by which, severe disease arises. Recent genomic sequencing and expression analysis tools have enabled the identification of the mechanisms that drive sex-specific gene expression and the effect this has on the embryo’s development. Single-cell sequencing data with transcriptomic profiling has demonstrated clear associations between sex chromosome complement and activation of developmental pathways which were traditionally poorly understood. Fundamentally, preimplantation research related to differential sex-specific expression would greatly improve genetic disorders linked to sex chromosomes. Particularly, X-linked diseases such as Hemophilia A and B, Hunter syndrome, and Duchenne-Becker MD. Genetic testing is usually only provided given the parents are at substantial risk of the genetic disorder. However, discussion remains for a practical alternative prenatal diagnostic and termination of pregnancy [18]. Indeed, the difficulty lies in the future development of preimplantation genetic diagnostic tools, to create technologies that are accurate, ethical, and legal [19].
References
[1] Feuer, S., & Rinaudo, P. (2012). Preimplantation stress and development. Birth Defects Research Part C: Embryo Today: Reviews, 96(4), 299-314.
[2] Ramathal, C., Pera, R. A. R., & Chavez, S. L. (2015). Preimplantation Embryo Development and Primordial Germ Cell Lineage Specification. Knobil and Neill’s Physiology of Reproduction: Two-Volume Set, 233-265.
[3] Saini, D., & Yamanaka, Y. (2018). Cell polarity-dependent regulation of cell allocation and the first lineage specification in the preimplantation mouse embryo. Current Topics in Developmental Biology, 128, 11-35.
[4] Knöfler, M., Haider, S., Saleh, L., Pollheimer, J., Gamage, T. K., & James, J. (2019). Human placenta and trophoblast development: key molecular mechanisms and model systems. Cellular and Molecular Life Sciences, 76, 3479-3496.
[5] Red-Horse, K., Zhou, Y., Genbacev, O., Prakobphol, A., Foulk, R., McMaster, M., & Fisher, S. J. (2004). Trophoblast differentiation during embryo implantation and formation of the maternal-fetal interface. The Journal of clinical investigation, 114(6), 744-754.
[6] Chazaud, C., Yamanaka, Y., Pawson, T., & Rossant, J. (2006). Early lineage segregation between epiblast and primitive endoderm in mouse blastocysts through the Grb2-MAPK pathway. Developmental cell, 10(5), 615-624.
[7] Richardson, V., Zhang, K., Engel, N., & Kulathinal, R. J. (2022). Comparative developmental genomics of sex-biased gene expression in early embryogenesis across mammals. bioRxiv, 2022-03.
[8] Hansen, P. J., Dobbs, K. B., Denicol, A. C., & Siqueira, L. G. (2016). Sex and the preimplantation embryo: implications of sexual dimorphism in the preimplantation period for maternal programming of embryonic development. Cell and tissue research, 363, 237-247.
[9] Deegan, D. F., & Engel, N. (2019). Sexual dimorphism in the age of genomics: how, when, where. Frontiers in cell and developmental biology, 7, 186.
[10] Werner, R. J., Schultz, B. M., Huhn, J. M., Jelinek, J., Madzo, J., & Engel, N. (2017). Sex chromosomes drive gene expression and regulatory dimorphisms in mouse embryonic stem cells. Biology of sex Differences, 8(1), 1-18.
[11] Winge, O. (1932). The nature of sex chromosomes. In Proc Sixth Int Congr Genet (Vol. 1, pp. 343-355).
[12] Mutzel, V., & Schulz, E. G. (2020). Dosage sensing, threshold responses, and epigenetic memory: a systems biology perspective on random X‐chromosome inactivation. Bioessays, 42(4), 1900163.
[13] Chaligné, R., & Heard, E. (2014). X-chromosome inactivation in development and cancer. FEBS letters, 588(15), 2514-2522.
[14] Fang, H., Disteche, C. M., & Berletch, J. B. (2019). X inactivation and escape: epigenetic and structural features. Frontiers in cell and developmental biology, 7, 219.
[15] Tarantino, D., Walker, C., Weekes, D., Pemberton, H., Davidson, K., Torga, G., … & NJ Tutt, A. (2022). Functional screening reveals HORMAD1-driven gene dependencies associated with translesion synthesis and replication stress tolerance. Oncogene, 41(32), 3969-3977.
[16] Zhou, Q., Wang, T., Leng, L., Zheng, W., Huang, J., Fang, F., … & Kristiansen, K. (2019). Single‐cell RNA‐seq reveals distinct dynamic behavior of sex chromosomes during early human embryogenesis. Molecular Reproduction and Development, 86(7), 871-882.
[17] Syrett, C. M., Sierra, I., Berry, C. L., Beiting, D., & Anguera, M. C. (2018). Sex-specific gene expression differences are evident in human embryonic stem cells and during in vitro differentiation of human placental progenitor cells. Stem Cells and Development, 27(19), 1360-1375.
[18] Braude, P., Pickering, S., Flinter, F., & Ogilvie, C. M. (2002). Preimplantation genetic diagnosis. Nature Reviews Genetics, 3(12), 941-953.
[19] Ginoza, M. E., & Isasi, R. (2020). Regulating preimplantation genetic testing across the world: a comparison of international policy and ethical perspectives. Cold Spring Harbor perspectives in medicine, 10(5), a036681.